Neutrino physicists spend a lot of time in the dark. As a figurative statement this reflects how difficult neutrinos are to understand, but it also reveals the literal sense that we work with experiments that do not see a lot of sun—and it’s not just the South Pole, it’s also in mines, tunnels, and deep underwater in seas and lakes. But just like a rare neutrino interaction, every so often a brief flash of light offers some new truth about the nature of our universe. Such a discovery moment was rewarded with this year’s Nobel Prize in Physics. Art McDonald and Takaaki Kajita were recognized for their contributions in establishing neutrino oscillations and confirming a non-zero neutrino mass, which is the first laboratory signal of physics beyond the Standard Model of particles and interactions.
After photons, neutrinos are the most numerous particles in the known universe. More numerous than all the atoms that make up all the stars, planets, black holes, dust, rocks, and so on. Up until the Higgs boson in 2013, the last observation of a previously missing particle in the Standard Model of particle physics was the tau neutrino in 2000, and that was done with the observation of only four tau-like neutrino interactions by the DONUT collaboration. The tau neutrino observation is an extreme example, but even the other two flavors of neutrino (muon and electron) are rarely detected compared to almost any other known particle. The reason that neutrino observations are so few, even though they are so numerous in the universe, is largely because they are electrically neutral, have an incredibly small rest mass, and have a low probability of collision with normal matter—basically the very properties you’d put together in a single particle if you wanted to drive experimentalists insane.
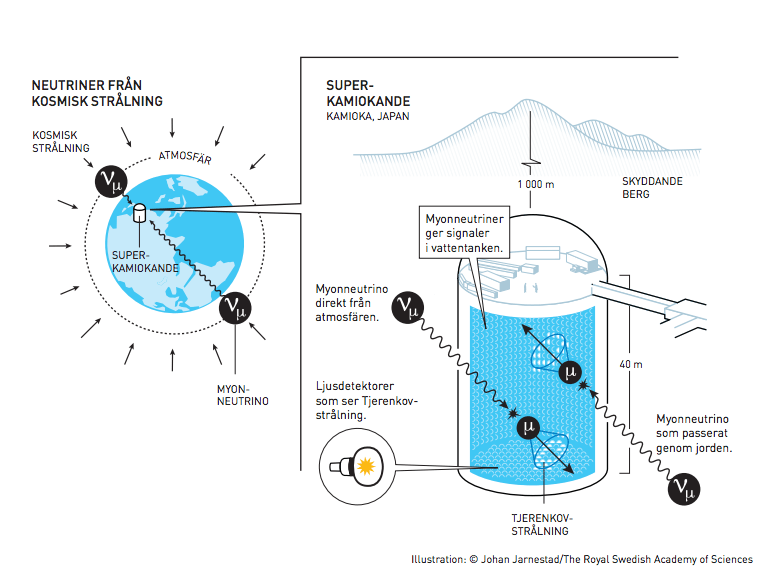
I was introduced to the world of neutrino oscillations in the summer of 2001 as an undergraduate student working on MINOS, a neutrino oscillation experiment that was then under construction. Not that I had noticed, but this was an important juncture because it was shortly after the seminal 1998 paper from Super-Kamiokande (Super-K), with strong evidence for atmospheric muon neutrino oscillation, and coincided with Sudbury Neutrino Observatory (SNO) publicizing their first solar neutrino oscillation results. At the time, there were some alternative theories on oscillations to explain the deficit of neutrinos seen from the Sun as well as a deficit from those produced in the Earth’s atmosphere. Obviously, oscillation was ultimately the solution for the respective neutrino deficit, but it was also a new conundrum. A core feature of the theory is that neutrinos must have a small, but non-zero, mass, which conflicts with the Standard Model prescription where they are massless by definition. So what started as a problem with neutrino rates evolved into one of the landmark discoveries of particle physics.
The electron-type neutrinos from the Sun had a similar issue to the atmospheric muon neutrinos, where the observed rate was lower than the predicted rate. The deficit had been detected by multiple neutrino experiments dating back to the 1960s, but could not be fully reconciled as the result of neutrino oscillation without extraordinarily thorough experimental verification. After more than a decade of planning and construction, SNO not only measured the features of the solar electron neutrino deficit, but also ingeniously verified that the total neutrino rate across all flavors (electron+muon+tau) was conserved. The precision of Super-K, led by Prof. Kajita, and the thoroughness of SNO, led by Prof. McDonald, were significant factors in establishing neutrino oscillations as the cause of the solar and atmospheric neutrino deficit.
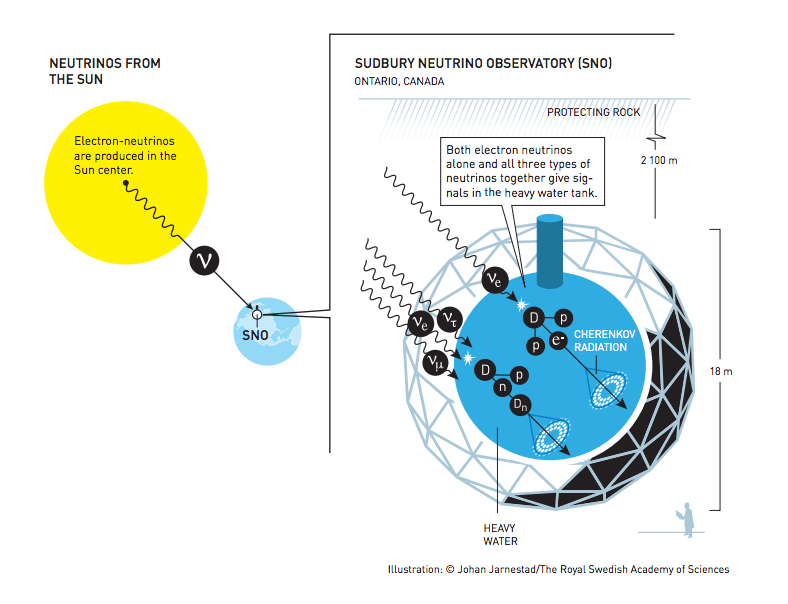
Even though I did not fully realize the impact of the oscillation results in 2001 (while I was sweeping and dusting an underground cavern in preparation for detector installation), I was learning that moments of “suboptimal” understanding happen rather frequently in neutrino research. You, or a colleague, make a plot that doesn’t seem right. The first reaction is to think that you, the researcher, introduced an error into the analysis; maybe a bug in your code or forgetting to divide by 2 somewhere. Then you might think that the detector is the one to be blamed, maybe that a sensor was not performing as expected. And most of the time an anomaly is the result of some correctable oversight. But not always.
It’s hard to imagine what goes through a researcher’s mind, or a group of them, when they finally realize that at-the-time anomalous results are not caused by detector or analysis failures, but because the Standard Model of particles and interactions is flawed and requires revision. It is certainly not something I thought about when I was first exposed to the transformative results. But I do wonder now what I would think, or do, if IceCube neutrino oscillation results required a non-conventional modification to fundamental physics.
I’m no longer an undergraduate student; instead I am a junior faculty member in the Niels Bohr Institute at the University of Copenhagen. Fourteen years have passed, all involved with oscillation research in some way, and I had hoped that by now I would be more knowledgeable about the neutrino and neutrino oscillation. But I feel like I’m just more aware of what is unknown. Are there only three neutrinos? Is there some underlying symmetry which governs neutrino oscillation? Why is the magnitude of neutrino mixing so much greater than quark mixing? What measurements are needed to confirm, or refute, the current model of oscillation among the three active neutrino flavors? Those questions and others are being tackled by an array of different experiments across the world, and IceCube happens to be involved, too.
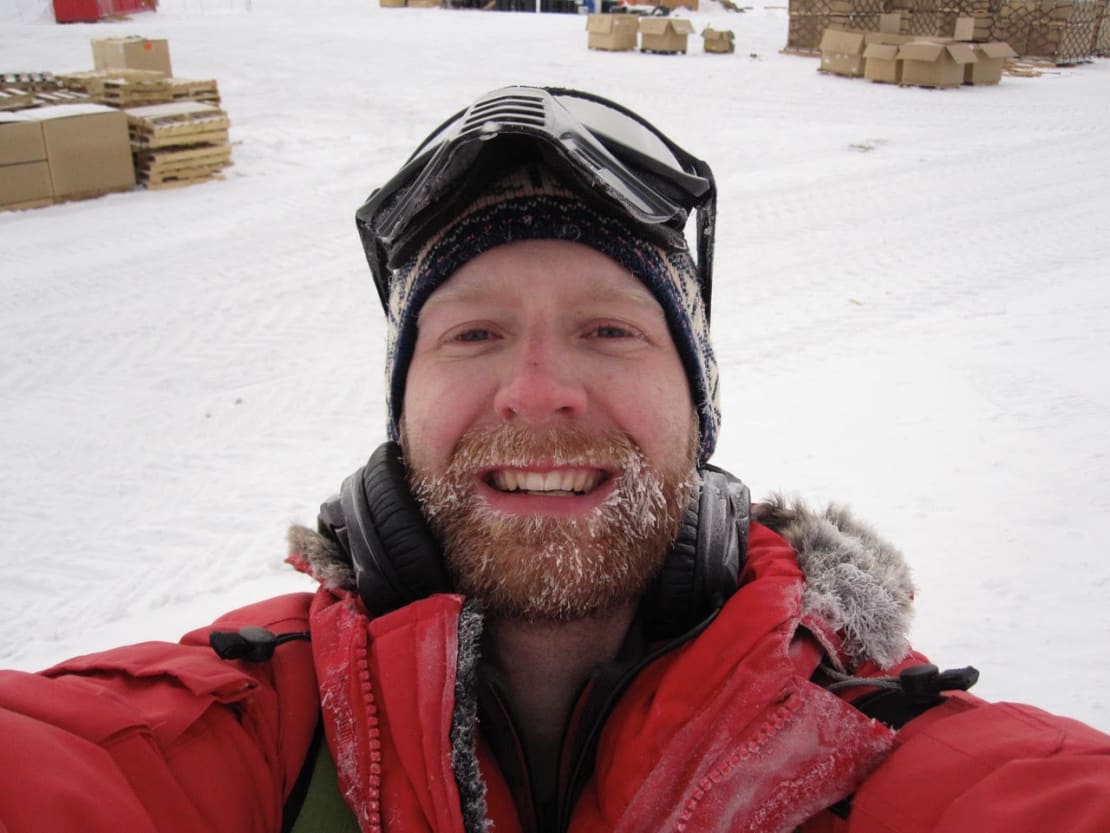
The IceCube Neutrino Observatory is pretty well known for its ability to examine the cosmos for high-energy neutrinos. With DeepCore, IceCube’s low-energy extension, the experimental sensitivity to make contributing atmospheric neutrino oscillation measurements was added in 2010/2011. What makes IceCube-DeepCore such a great tool for studying oscillations is that it is big, really big. Because neutrino interactions are so rare, being big translates into collecting one of the largest atmospheric neutrino data samples in the world. Using the natural source of neutrinos produced in the atmosphere to make oscillation measurements, similar to the original Super-K oscillation result, offers complementarity and competition to the other global experiments that nowadays make extensive use of manmade neutrino beams. Our most recent atmospheric muon neutrino oscillation results, published in 2015, approach the sensitivity of other dedicated oscillation experiments. This is impressive when one considers that the result uses only three years of IceCube-DeepCore data from a still maturing analysis chain. With more data, better reconstructions, and enhanced event selections, I anticipate that IceCube-DeepCore results will transition from being supporting to being competitive and then to becoming world-leading in upcoming iterations.
Physicists talk about neutrino “disappearance” or “deficit,” but really a deficit just conveys that the neutrino is oscillating to a flavor other than the one it started as and frequently to a flavor that the experiment or analysis is insensitive to. The counterpart to a neutrino deficit from oscillation is neutrino appearance. For IceCube-DeepCore, an important transition that is extremely difficult for other experiments to probe is “tau neutrino appearance,” whereby a muon neutrino oscillates to a tau neutrino. The experimental issue is that the tau neutrino has a much lower interaction probability than the already quite low muon and electron neutrinos, at least at the energies relevant for atmospheric oscillation. Additionally, in IceCube-DeepCore a tau neutrino collision is difficult if not impossible to separate from other types of nonsignal neutrino interactions. But once again, being big means having thousands of tau neutrino collisions in our data each year. While other leading experiments such as OPERA and Super-K are reaching their limits in measuring oscillation parameters via tau neutrino appearance, IceCube-DeepCore is just getting started.
For IceCube, being big means more than just detecting lots of atmospheric neutrinos (and as of 2013 astrophysical neutrinos, too). Being big opens up neutrino research in energy regions that cannot be experimentally probed by any other experiment. One such example is that we collect the largest number of neutrinos at energies more than 100-1,000 higher than where conventional atmospheric oscillation can be observed. In this energy region, we search for new oscillation effects from exotic theories that predict more than the three Standard Model neutrinos.
Ultimately, there is a limit to what the current IceCube detector is capable of for probing neutrino properties via oscillation, even with the low-energy DeepCore extension. In order to reach new topics such as nonmaximal neutrino mixing or resolving the neutrino mass ordering, there is a push to deploy the Precision IceCube Next Generation Upgrade (PINGU) as a part of the IceCube-Gen2 effort. While the Nobel Committee honors the past, we are planning for the future.
Besides not being recognized by Nobel Prize related news for my indispensable work dusting and sweeping an empty MINOS detector hall as a student, my biggest issue is that the work led by Art and Kajita violates the cardinal rule of “you break it, you fix it.” Unlike the Higgs boson discovery, which provided a beautiful solution to generate masses for the Standard Model particles (except those pesky neutrinos, of course), neutrino oscillation and confirmation of a non-zero neutrino mass took a nice elegant picture of fundamental particles and messed it up. Personally, though, I couldn’t be more grateful that it happened, because although Profs. McDonald and Kajita have received a prize, the wider physics community (including IceCube) gets the gift of a better understanding of a basic building block of our universe. Thanks to them and their colleagues, life as a neutrino physicist is a little less dark now.