The IceCube Neutrino Observatory is the first, and so far the only, cubic-kilometer neutrino telescope. IceCube is a flagship experiment in neutrino and multimessenger astronomy thanks to the discovery of very high energy cosmic neutrinos and the detection of the first likely source of high-energy neutrinos, a blazar that was also observed with gamma rays and lower energy photons. IceCube is also a multipurpose research facility with outstanding precision measurements in neutrino physics and exceptional contributions to cosmic ray physics, dark matter searches, and glaciology.
![]() | Neutrino Astronomy and Multimessenger Astrophysics | |
![]() | Cosmic Ray Physics | |
![]() | Neutrino Physics | |
![]() | Dark Matter | |
![]() | Glaciology |
Neutrino Astronomy and Multimessenger Astrophysics
The IceCube project transformed a cubic kilometer of transparent natural Antarctic ice into a Cherenkov detector that observes neutrinos from GeV to PeV energy. IceCube discovered PeV-energy neutrinos originating beyond our galaxy with an energy flux that is comparable to, and apparently exceeds, that of high-energy gamma rays of extragalactic origin. The first high-energy astrophysical neutrino flux revealed by only two years of data in 2013 provided an unparalleled, unobstructed view of the cosmic ray accelerators that power the highest energy radiation reaching us from the universe.
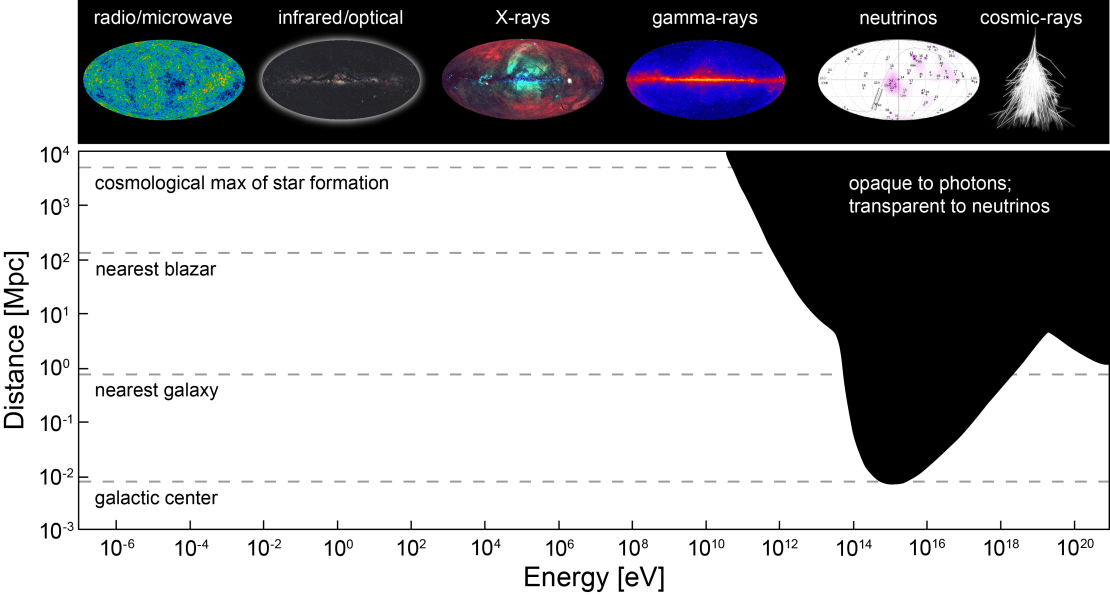
The highest energy neutrinos observed by IceCube so far have energies more than a thousand times those produced with earthbound accelerators and a billion times those detected from supernova SN1987 in the Large Magellanic Cloud, the only neutrinos detected on Earth from outside the solar system prior to IceCube’s breakthrough. The most surprising property of these cosmic neutrinos is their large flux. The photon flux from the decay of neutral pions, that inevitably accompany the charged pions that are the parents of cosmic neutrinos, exceeds the extragalactic high-energy gamma-ray flux observed by the Fermi Gamma-ray Space Telescope. There is no contradiction here—the photons lose energy in the dense sources, which are efficient at producing the neutrinos, and arrive at Earth with MeV energies or below. This is confirmed by IceCube’s observation of the first sources, such as NGC 1068, discussed below.
The flux of cosmic muon neutrinos (shaded band) and electron and tau neutrinos (data points). Both are consistent with the flux derived from the observation of a single Glashow-resonance event (red data point), where an antielectron neutrino interacts with an atomic electron to produce a charged intermediate boson W.
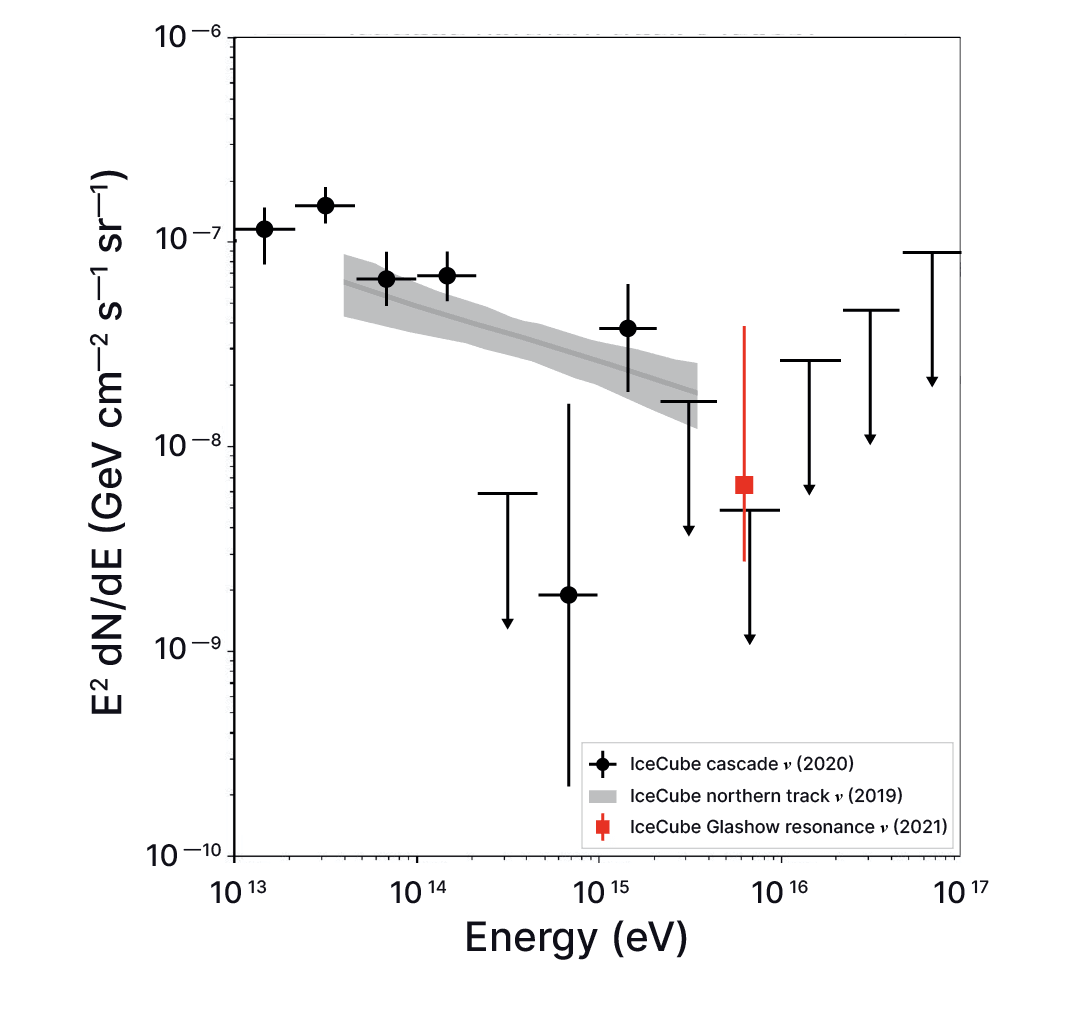
Also surprising is the fact that, unlike the case for observations of electromagnetic radiation of any wavelength, the neutrino sky map is not dominated by nearby sources in our own galaxy, as would be expected from simple geometry. Powerful extragalactic sources outshine the neutrino sources in the Milky Way, which represent a subdominant component in the map. This galactic flux has recently been observed by IceCube at the level of 10% of the extragalactic flux. It originates from interactions of cosmic rays with the interstellar medium, and the measured neutrino flux agrees with the diffuse photon flux of galactic origin observed by Fermi at lower energies.
After having collected a decade of data with the completed IceCube detector, we searched a high-purity sample of 670,000 muon neutrinos for sources of astrophysical origin. Their arrival directions reveal the most significant excess, 80 neutrino events of TeV energy, to be within 0.18 degrees of the active galaxy NGC 1068 (M77). NGC 1068 is also the most significant astrophysical neutrino source identified from a search at the positions of 110 preselected high-energy gamma-ray sources. A search for subdominant sources in the sky map reveals evidence for two more active galaxies, PKS 1424+240 and TXS 0506+056. TXS 0506+056 had already been identified as a neutrino source from a multimessenger campaign triggered by an IceCube-detected neutrino of 290 TeV energy as well as from the observation in archival IceCube data of an earlier neutrino burst from TXS 0506+056 in 2014-15. The multimessenger campaign involved follow-up observations by gamma-ray, X-ray, and optical telescopes that were triggered by a real-time neutrino alert from IceCube on September 22, 2017.
The data point at the obscured dense cores near the supermassive black holes of some active galaxies, typically within 10~100 Schwarzschild radii, as the site where cosmic rays are accelerated and neutrinos produced. As a result, the gamma rays that inevitably accompany cosmic neutrinos rays lose energy in the obscured core and emerge with MeV energies or below.
Neutrinos have thus given us a first glimpse at the still enigmatic cosmic accelerators where high-energy cosmic rays are produced
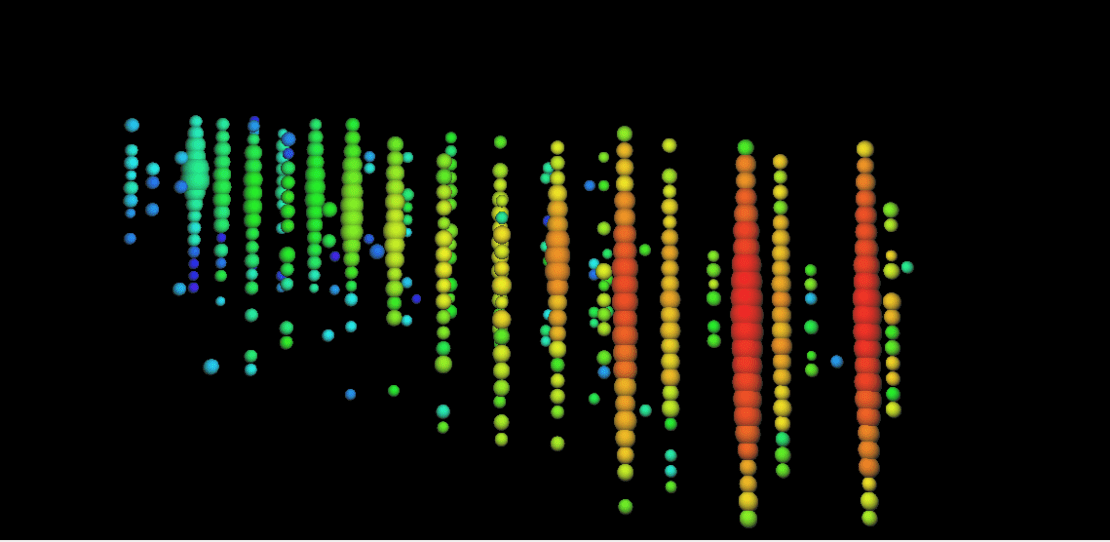
IceCube has developed a powerful real-time follow-up program that targets transient sources. This multimessenger program sends alerts of the arrival direction of single high-energy events or clusters of lower energy neutrino events (multiplets) less than one minute after their detection. In collaboration with other observatories, we aim to identify the electromagnetic counterpart of a rapidly fading source or coincident gravitational waves. Single event alerts are distributed publicly as GCN alerts, while multiple alerts are distributed through individual agreements with optical, X-ray, and gamma-ray observatories. Searches for bursts of much lower energy neutrinos from nearby supernovas are also performed continuously in real time, and any detections are automatically announced within the SNEWS network.
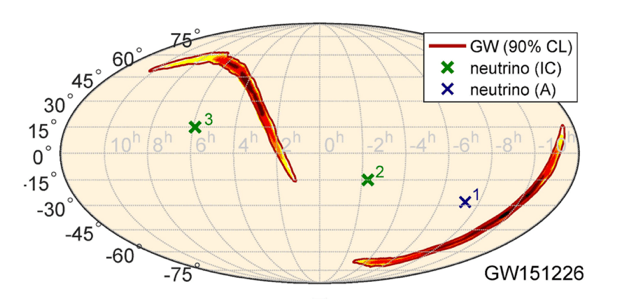
We have also conducted searches for the so-called cosmogenic neutrinos produced in the interactions of cosmic rays with microwave photons. The characteristic energy of these extragalactic neutrinos is in the EeV range, but so far we have not observed a neutrino with an energy of more than 10 PeV. IceCube currently has the world’s best limit on the flux of cosmogenic neutrinos, which places strong constraints on the properties of the sources of ultra-high-energy cosmic rays. Proton-dominated sources are disfavored, and the data point to a heavier composition, in agreement with the results from direct high-energy cosmic array observations.
Cosmic Ray Physics
Beyond its primary purpose as a neutrino telescope, IceCube is also an excellent detector for cosmic rays—high-energy protons and atomic nuclei from beyond our solar system. IceCube’s surface array, IceTop, measures millions of atmospheric particle cascades in the energy range of 1014 to 1018 eV, so called air showers, originating from cosmic-ray collisions with the atmosphere. In addition, the deep optical array registers more than 70 billion muons per year in the ice, produced in such air showers.
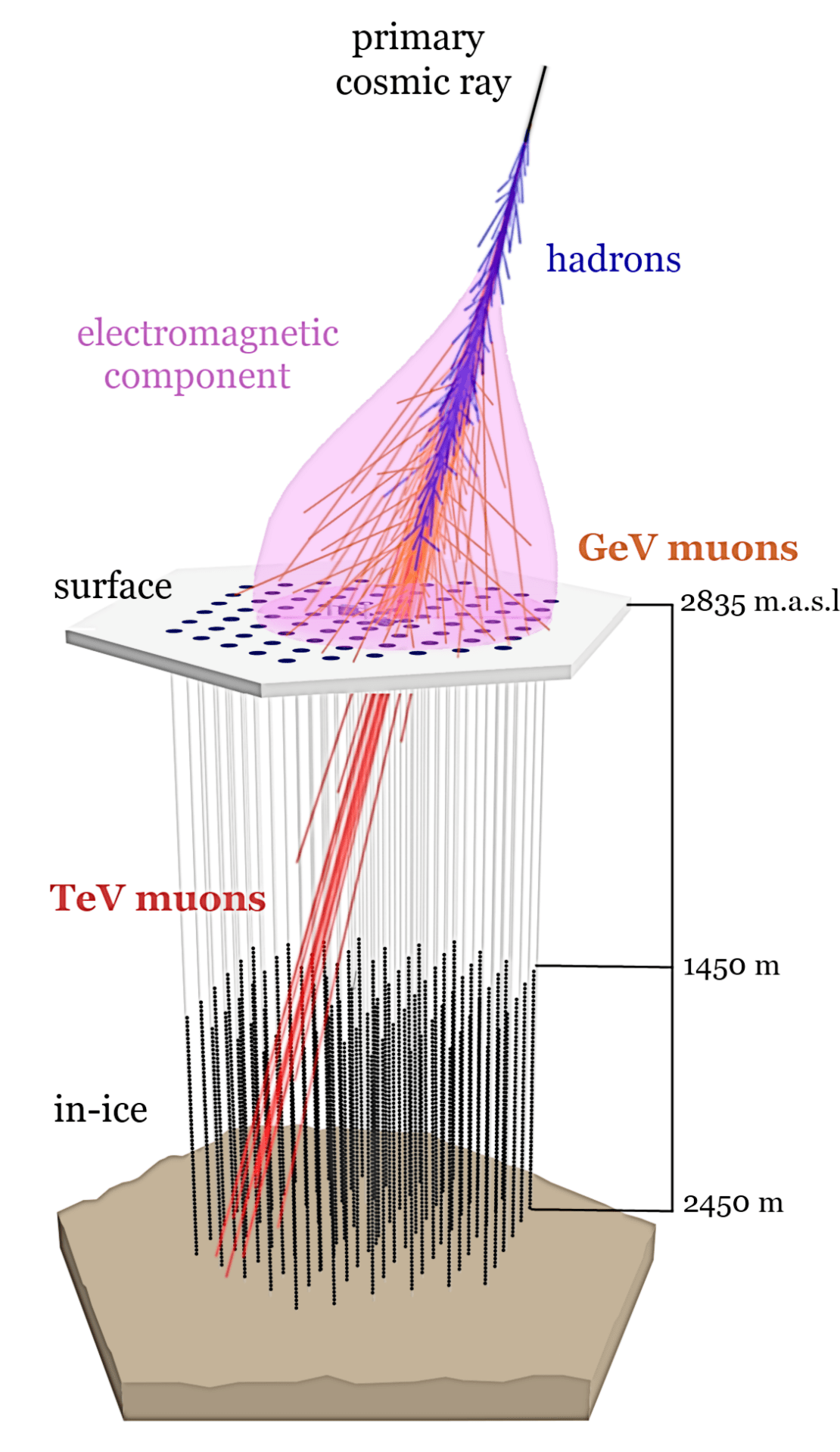
The combination of the in-ice and surface detector makes IceCube a unique laboratory for the particle physics in these air showers, as demonstrated below. This setup has been used to investigate key questions in the field, such as a yet to be understood mismatch in the muon number predicted by state-of-the-art hadronic interaction models and air-shower measurements at the highest energies and the flux of so-called prompt muons and neutrinos originating from the decay of particles with heavy quarks that are produced in high-energy atmospheric collisions.
Furthermore, IceCube measures the energy spectrum and chemical composition of galactic cosmic rays. These measurements are crucial input for more precise calculations of the expected flux of atmospheric neutrinos, and they enable testing of astrophysical scenarios for the still unknown sources of the most energetic cosmic rays in our galaxy. IceCube confirms deviations of the cosmic-ray energy spectrum from a simple power law, such as the “second knee” above 1017 eV, which could be the starting point of the transition from galactic to extragalactic cosmic rays at higher energies.
Another way to learn about the origin and propagation of galactic cosmic rays is the measurement of small anisotropies in the arrival direction of cosmic rays. IceCube revealed such anisotropies from the per-mille down to the 10-5 level and measured significant structures on multiple angular scales, as shown below. Moreover, the structure and phase of the anisotropies changes with energy, and the collaboration with the HAWC observatory in the Northern Hemisphere enabled the production of a full-sky anisotropy map. Finally, IceCube has discovered anisotropies up to energies beyond 1015 eV, higher in energy than any other experiment for galactic cosmic rays.
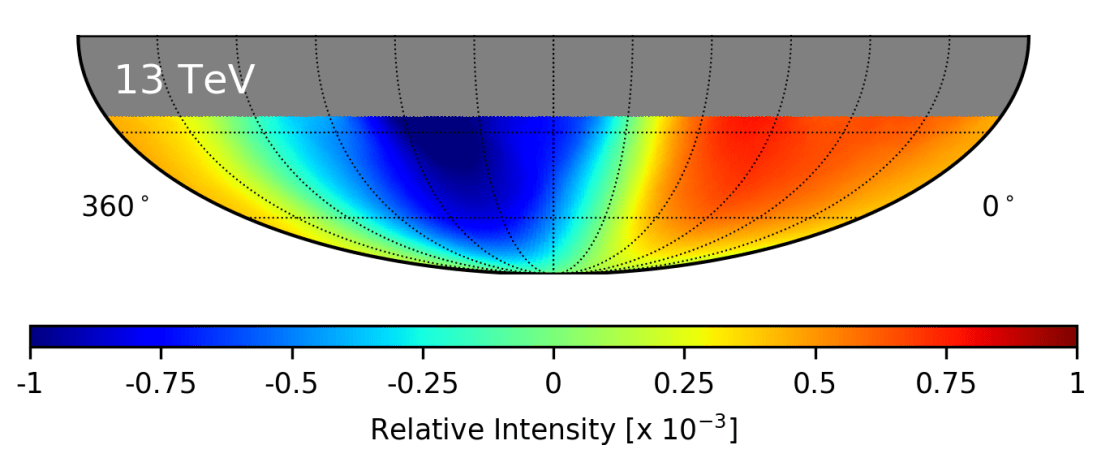
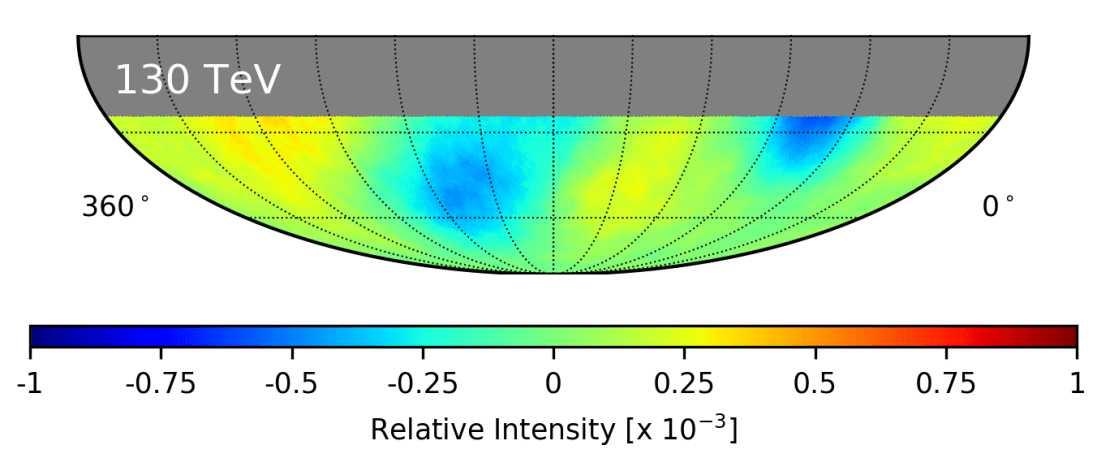
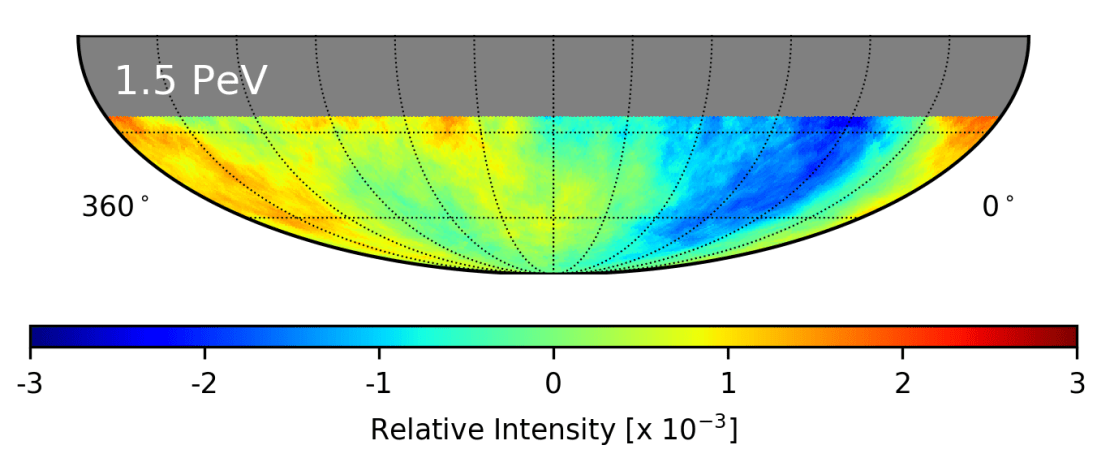
Finally, IceCube’s cosmic-ray physics contributes to other areas of science. Examples are atmospheric physics, as the seasonal variations measured in the neutrino and muon rates depend on the conditions in the stratosphere, and solar-heliospheric physics through the measurement of solar events with the IceTop detectors.
Neutrino Physics
IceCube has recorded an unusually large set of neutrinos. From over ten years data, we have accumulated about 700,000 between 100 GeV and 1 PeV and 200,000 between 10 GeV and 100 GeV. Both datasets have outstanding purity, at more than 99% with respect to downgoing cosmic ray muons. This wealth of data covering such a wide range of energy enables IceCube to conduct detailed studies of neutrino properties, such as atmospheric neutrino oscillations, sterile neutrinos, nonstandard neutrino interactions, quantum gravity effects on neutrino oscillations, among others. Here we summarize two: muon neutrino disappearance in the tens of GeV energy range and the search for sterile neutrinos in the TeV scale.
Studies in the 10-100 GeV energy range rely on DeepCore, the densely instrumented subarray within the full IceCube detector. The higher density of photocathode area lowers the detector threshold from 100 GeV for the main IceCube array to 10 GeV for DeepCore. The measurement of oscillations with DeepCore is complementary to accelerator-based observations. Because oscillations depend on the ratio of distance to energy, to observe them over the diameter of Earth, IceCube uses neutrinos of energies higher than accelerator experiments, which have baselines of hundreds of kilometers. One fantastic advantage of working at higher energies is that tau neutrinos have an energy that is higher than the tau-lepton production threshold, allowing the observation of tau neutrino appearance to be done with DeepCore.
Current state of the art in the use of DeepCore is the suite of studies referred to as OscNext. Compared to previous DeepCore work, OscNext analysis benefits from improved background rejection, new simulation with updated modeling of the optics of the ice, new reconstruction, a new machine learning particle classification algorithm, and improved modeling of systematic uncertainties. Using OscNext to search for muon neutrino disappearance on atmospheric neutrino data results in a sensitivity to Dm322 vs. sin(q23) that is world leading.
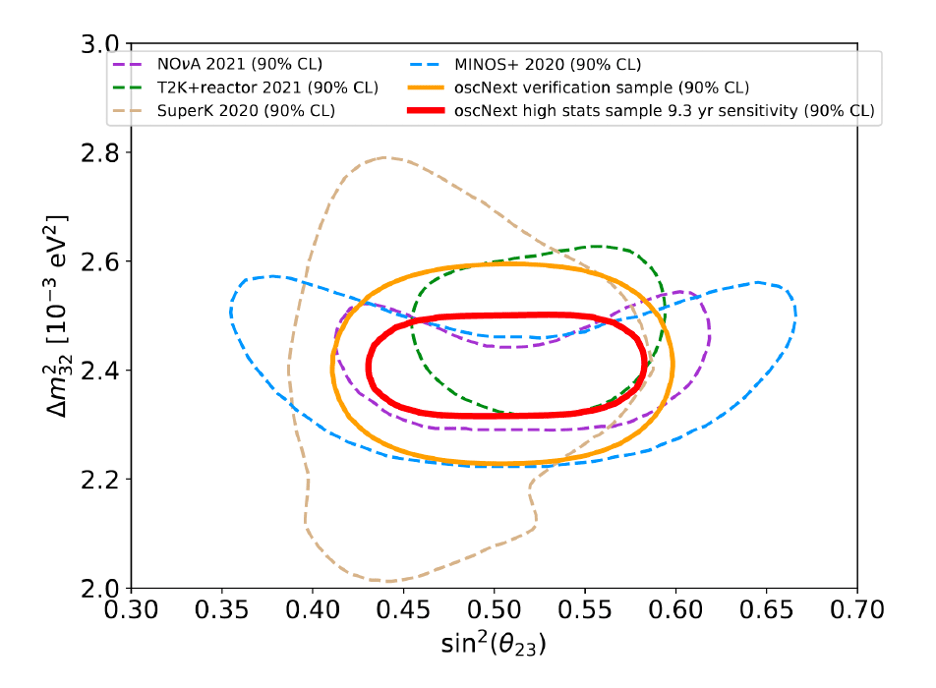
Oscillation parameters measurements anticipated from the analysis of the new 9.3-year data sample OscNext. A sensitivity test yields the 90% expected contour (in red), which is compared to the 90% confidence level expected for an analysis using well-reconstructed events only (OscNext verification; gold) and to other experiments’ published results (dashed lines).
Over the past two decades, several experiments have reported results that cannot be easily reconciled with a three-neutrino flavor scenario. The existence of a fourth neutrino, called “sterile” because it would not interact via the weak force, has been suggested to resolve this apparent discrepancy. The high statistics and wide energy coverage of IceCube implies that a search for sterile neutrinos is not only possible but also highly impactful. The most recent study by IceCube for eV-scale sterile neutrinos uses eight years of data. Most of the sensitivity to sterile neutrino by IceCube is on TeV-scale and depends on matter-enhanced resonance. This resonance results in the almost complete disappearance of atmospheric muon neutrinos over a very small energy range, and “standard” oscillations do not significantly change this energy range. As a result of this work, IceCube can set world-leading constrains on the existence of sterile neutrinos.
Results from the IceCube search for light sterile neutrinos using high-energy atmospheric neutrinos. The 90% and 99% C.L. contours, assuming Wilks’ theorem, are shown as dashed and solid bold blue lines, respectively. The green/yellow band shows the region where 68%/95% of the pseudoexperiment’s 99% C.L. observations lie; the dashed white line corresponds to the median. Other muon-neutrino disappearance measurements at 99% C.L. are shown in black. The star marks the analysis’s best-fit point location.
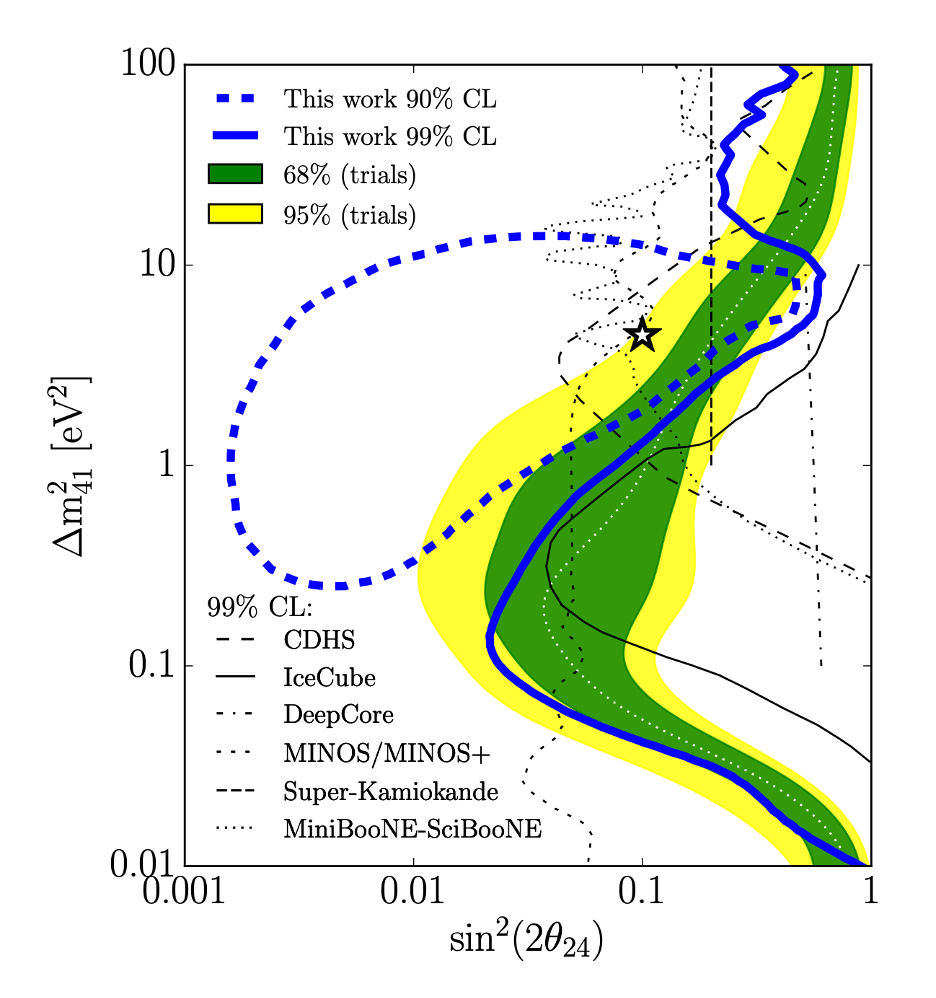
Dark Matter
The nature of dark matter is one of the greatest mysteries of contemporary physics. From cosmological and astrophysical observations, we know that approximately 95% of the matter in the universe is dark matter. IceCube has multiple ways to uniquely shine light on the nature of dark matter.
One possibility is that dark matter annihilates, in other words, collides with another dark matter particle, or disintegrates producing Standard Model particles. From the decay of these particles, neutrinos and other cosmic messengers are produced. IceCube has searched for excess neutrinos from regions where a large accumulation of dark matter is expected, such as the Earth’s core, the Sun, the Galactic Halo, or galaxy clusters. These searches have placed world-leading constraints on the spin-dependent cross section and the lifetime of corpuscular dark matter. The scenario mentioned above is motivated by supersymmetric models where the neutralino is the usual candidate dark matter particle. Additionally, these scenarios are also motivated by scotogenic neutrino mass generation models. In scotogenic models, neutrino mass—whose origin is currently unknown—is produced by the interaction of dark matter with neutrinos. In some of these scenarios, such interactions also produce the annihilation of dark matter into neutrinos.
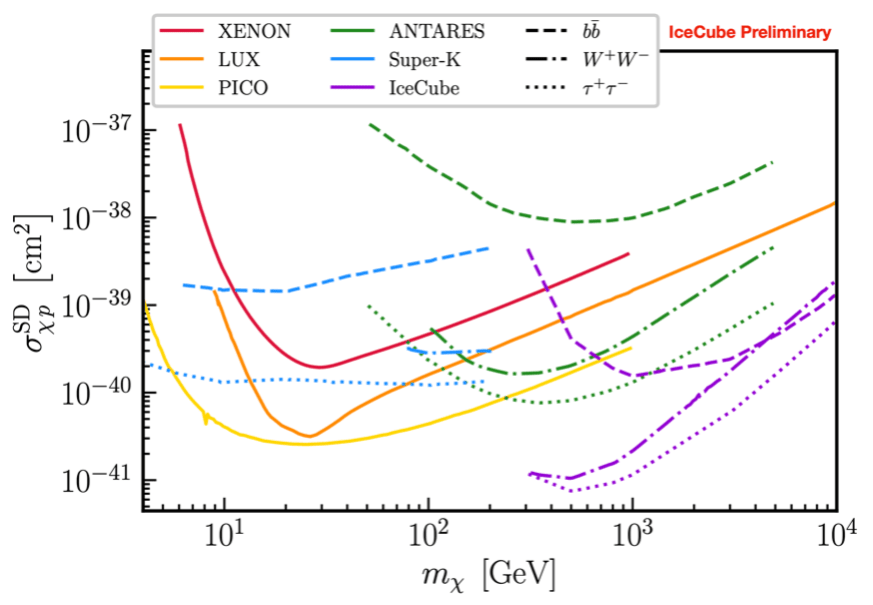
Shows the 90% confidence level upper limits on the spin-dependent (SD) cross section for dark matter annihilating into hard (W+W–, tau+-) and soft (b bar b) channels. For comparison, constraints from other neutrino detectors (ANTARES, Super-K) are shown as well as from dark matter direct detection experiments (XENON, LUX, PICO).
Another interesting possibility, motivated by scotogenic neutrino mass scenarios, is that high-energy neutrinos scatter with dark matter present in the universe. As neutrinos travel from extragalactic sources, such as NGC 1068 or TXS 0506+056, they can interact with dark matter that permeates spaces. This interaction produces unique signatures that can be searched for in the neutrino spectra observed on Earth. The most striking signature is the predominant disappearance of neutrinos in the direction of the galactic center, where the number of dark matter particles that the neutrinos have to traverse is the largest. IceCube has performed a search for this signature using the highest energy neutrinos that interact within our detector and put some of the strongest constraints in the interactions between neutrinos and dark matter. These constraints are complementary to cosmological probes that look for similar interactions in the early universe.
Glaciology
To realize the full potential of IceCube, light propagation in the glacial Antarctic ice must be well understood, which requires in situ measurement since natural glacial ice is unlike any laboratory ice. IceCube makes these measurements utilizing LED light sources installed on board its sensors, the deepest camera system ever deployed in ice, along with a borehole laser logger.
The laser logger provided a millimeter-resolution stratigraphy of impurities, which allowed us to reconstruct a detailed climate record of the last glacial period. We found evidence for the Toba volcano eruption 74,000 years ago, which had never been observed in ice-core studies. These results helped establish the South Pole as the site for a major American ice coring mission, the South Pole Ice Core Project. The laser logger also mapped out the undulation of ice isochrons (layers of equal age and optical properties), which primarily trace the topography of the underlying bedrock and can reach slopes of up to 10% across IceCube.
Comparison of data from the LED light sources to high-throughput simulations is the primary tool to convert the high-resolution stratigraphy into absolute optical properties. This established the low-impurity ice in the depth range instrumented by IceCube as the least optically absorbent solid known to date.
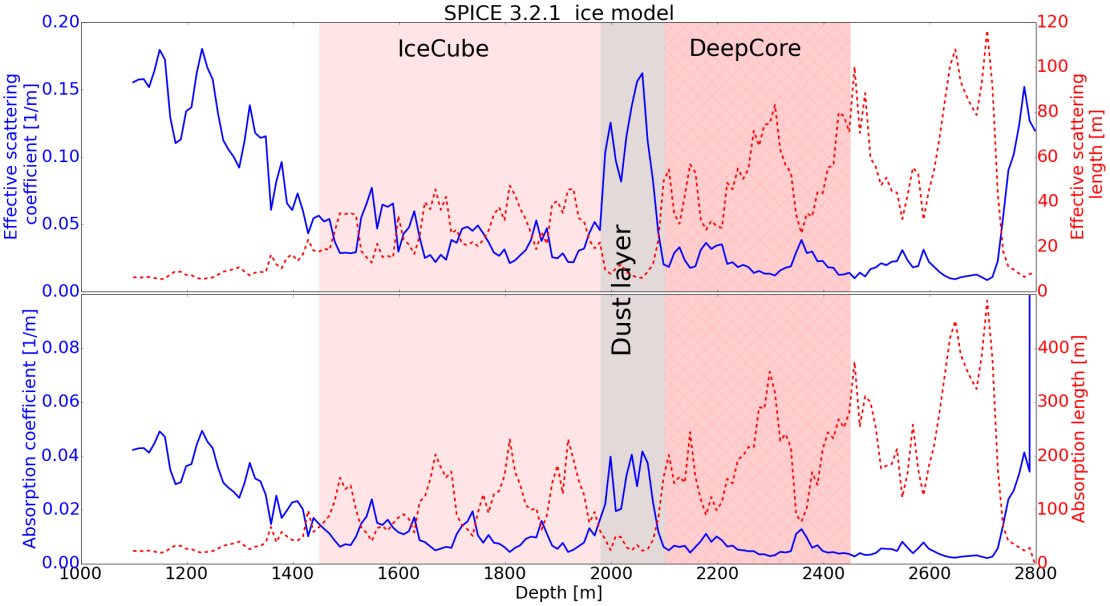
A surprising discovery made only after the deployment of IceCube was that light preferentially propagates in the direction of the glacial flow. This effect is now understood to be the result of curved light trajectories resulting from an asymmetric diffusion in the birefringent polycrystalline microstructure of the ice itself.
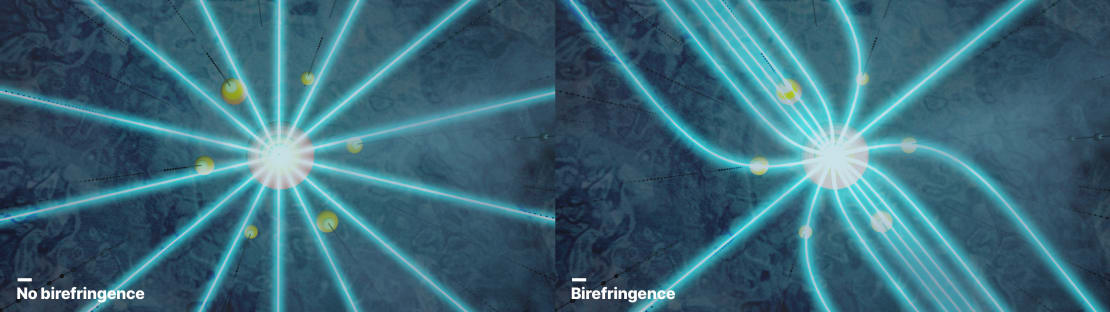
As the neutrino science analyses mature, the demands on the precision of the optical modeling keep growing. For this reason, the upcoming IceCube Upgrade features a large number of purpose-built calibration devices, including both fixed-focus and adjustable-focus cameras, isotropic and collimated light sources, and steerable light sources.