There are over 5,000 eyeballs buried in the ice at the South Pole. Not real eyeballs, but basketball-sized devices called digital optical modules (DOMs) that serve as the eyes of the IceCube Neutrino Observatory. Each DOM contains an instrument called a photomultiplier tube (PMT) that, like your eye, is able to register small amounts of light. Unlike your eye, these devices can register single photons that can be collected and sent to computers for processing.
IceCube’s eyes are looking for signs that elusive, subatomic particles called neutrinos are passing through the ice. Specifically, IceCube pursues neutrinos that originate far out in the universe: astrophysical neutrinos. Sometimes, when an astrophysical neutrino passes through the detector, it interacts with matter near the detector and produces a cone of light called Cherenkov radiation. When a particle of that light—a photon—hits the surface of a DOM, its minute interaction can be amplified by the PMT into a signal that the electronics are able to register.
Researchers in the IceCube Collaboration are always looking for ways to improve the understanding of the PMTs so they can get the highest-quality data from the DOMs. Most recently, they implemented a new method for more accurately characterizing individual PMT charge distributions, which was shown to improve PMT calibration and simulation. The method is described in a technical report, “In-situ calibration of the single-photoelectron charge response of the IceCube photomultipliers,” submitted recently to the Journal of Instrumentation.
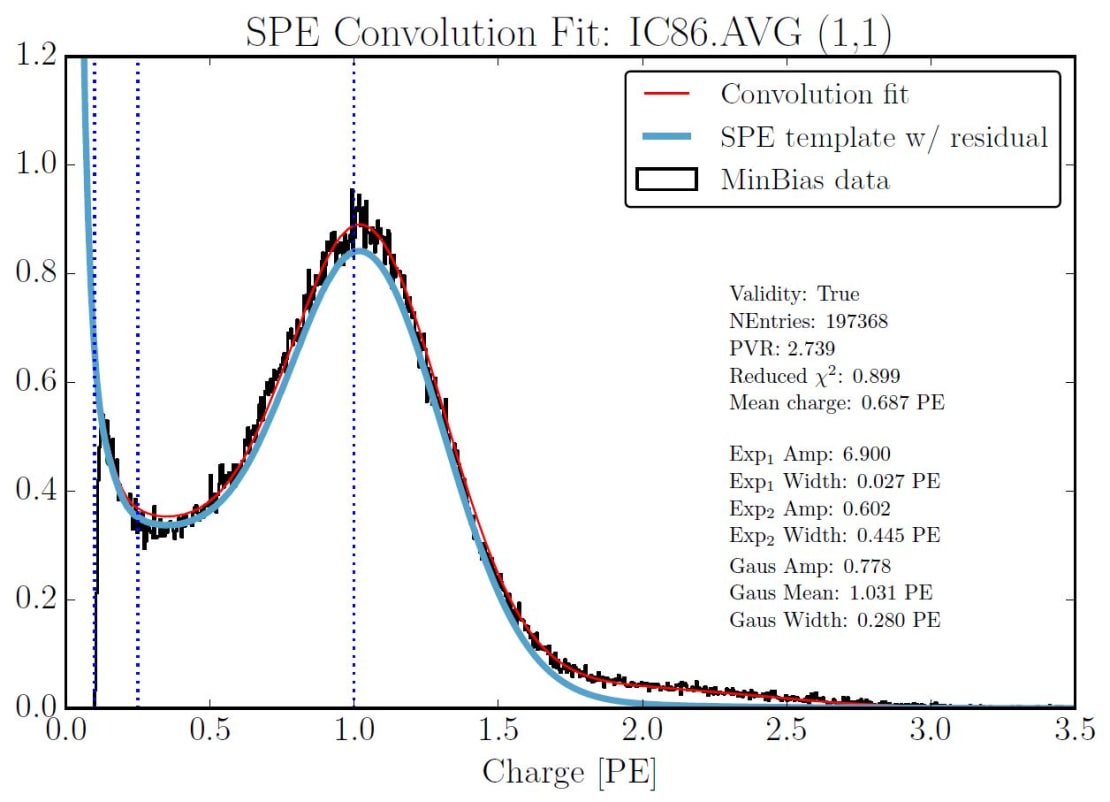
When a photon of Cherenkov light reaches a PMT inside a DOM, it will first encounter the photocathode, which in turn ejects an electron. That electron will be attracted to a charged plane (dynode) inside the PMT that then releases several new electrons. These electrons are attracted by the next dynode, which ejects even more electrons, and so on. This continues until an avalanche of electrons hits the final plane (anode). The pulse of charge generated by this avalanche is then digitized and the electrical output is sent up through a cable to the IceCube computing laboratory. The PMT’s ability to amplify the signal from photocathode to anode is known as its “gain.”
Many factors make it difficult to determine the actual gain on an individual PMT, and using the wrong gain in simulations can cause problems. This has happened in previous IceCube analyses, leading to small but detectable disagreements between the simulation and the experimental data, especially in charge-related variables such as the average charge collected per PMT, per event. Since simulations are used to analyze IceCube data, it is important to find the accurate gain for PMTs.
But no two PMTs are exactly the same. So researchers express the expected charge that a single photon generates in a given PMT as a probability curve, known as the single photoelectron (SPE) charge distribution. (Observed charge is often rescaled in units of photoelectrons.) The measured gain can also be used for evaluating the long-term stability of the detector and the accuracy of previous calibrations.
One of the problems the researchers had to address was contamination in the signal. “A single photon produces a single electron at the photocathode,” explains Spencer Axani of the Massachusetts Institute of Technology, who led this analysis. “This electron then gets amplified, and the average resulting charge, about 107 electrons, is what we call an SPE. But the amplification is a statistical process, and there are a lot of physical processes that can lead to additional smearing of the amplified charge of an individual photon.”
So Axani and his collaborators used a specially designed pulse selection software to remove some forms of contamination, allowing them to extract a sample of single photoelectrons from the data. They then used a newly developed fitting algorithm to create an SPE charge template per DOM.
This extraction of SPE distributions allowed for an improved description of the detector in simulation and several interesting studies. For example, Axani and his collaborators inserted the SPE charge templates into simulation, which improved the data-simulation agreement in charge-related variables. This led to accurate determination of the gain setting and therefore allowed researchers to confirm that previous calibration had been done properly. The more accurate gain will also improve future calibration.
They also used the measured distributions to search for changes in the fitted quantities (for example, what fractions of photons get properly or poorly amplified by the PMTs) over time. No changes were observed, which indicates that the calibration of the detector is properly accounting for the slow aging of the PMT.
Lastly, researchers used the SPE charge template to investigate correlations between the measured charge distribution from each DOM and their corresponding hardware. The IceCube detector includes two sets of DOMs—high quantum efficiency and standard efficiency DOMs—and Axani and his collaborators found that the shape of the SPE charge templates for these two subsets are somewhat different. Now, their differences have been modeled.
“Overall, we are pleased with the improvement that we saw in the agreement between the experimental data and the simulated data for charge-related variables,” says Martin Rongen of RWTH Aachen University, another lead on this analysis. He and Axani say their method can also be used to more accurately set the gain on the in-ice PMTs in future data-taking periods, thus improving the overall calibration of the detector.
info “In-situ calibration of the single-photoelectron charge response of the IceCube photomultipliers,” IceCube Collaboration: M. G. Aartsen et al., Journal of Instrumentation 15 (2020) P06032, iopscience.iop.org, arxiv.org/abs/2002.00997